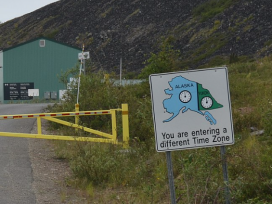
Clock times and social times conflict across much of Europe. Timekeeping is a deeply political matter, and João Lipinsky Nunes of Better Times takes issue with them.
The number of deaths caused globally by antibiotic resistance is increasing at alarming speed. The problem is well known, but governments and pharma appear not to take it seriously. Progress in bacteriophage research gives cause for optimism, however: using CRISPR gene editing technologies, these organisms can be deployed as an alternative to antibiotics.
In November 2015 Tom Patterson, a professor of psychiatry at the University of California, was on holiday with his wife Steffanie Strathdee when he fell ill. Patterson, who is now 70, had a gallstone and a pancreatic abscess, both of which were treatable. But an infection by Acinetobacter baumannii caused a greater problem. This organism, which colonises skin wounds such as burns, became notorious for its resistance to antibiotics during the 2003 Iraq war. Patterson became comatose and increasingly skeletal; the antibiotics he was given were failing. But Strathdee is an infectious diseases epidemiologist and she knew something about bacteriophages, microscopic organisms that had been used to combat infections successfully in the Soviet Union and other parts of Eastern Europe for a century but had never been accepted by Western medicine. Bacteriophages, or “phages” as they are commonly known, were allowed only as a last resort in cases like Patterson’s.
Strathdee mobilised the search for a phage that targeted A. baumannii. Ten phages were known to do this but none worked on Patterson’s sample. So they had to go fishing – in effluent from sewage-treatment plants; in ponds; in swabs from pigs on ranches near Patterson’s university. Phages lurk in the murkiest of places. Out of 126 samples, three were effective against Patterson’s strain of Acinetobacter. The US Food and Drug Administration granted emergency approval for a non-licensed therapy. Treatment began in March 2016 and Patterson made a successful recovery.
Yet why was such a desperately needed treatment only available to Patterson as a last resort? Each year, according to a 2016 UK government report, around 700,000 people around the world die because bacteria are becoming more resistant to antibiotics; by 2050 this figure could rise to 10 million. England’s chief medical officer, Sally Davies, has warned that “we may all be back in an almost 19th-century environment where infections kill us as a result of routine operations.” But although everyone is talking about it, there is no sign that governments and the pharmaceutical industry are prepared to take serious action. How did we get to this point, and could phages be the future?
In the golden age of antibiotics – the 1950s and 1960s – new ones appeared fast enough to keep the problem at bay. But now, new antibiotics are rare and clinicians have resorted to bringing back older, less safe varieties. In 1980, 25 large pharmaceutical companies were making antibiotics; now just four do. Antibiotics make no profit and Big Pharma has largely pulled out of the market. There are plenty of small, eager companies doing valuable research but they need the industry giants to take over when a promising drug is found. Foundations such as Wellcome and Gates are active in the field, but even they don’t have the financial muscle to solve the problem alone.
A current temporary solution is to modify existing antibiotics with an accessory drug that can attack the resistance-causing mechanism in bacteria. For example, while writing this article, I was hospitalised and needed antibiotics for a post-operation infection. I was given amoxicillin, a general-purpose antibiotic that has been heavily compromised by resistance, but there was a twist: in my version the amoxicillin was combined with clavulanic acid, which prevents resistant bacteria attacking the amoxicillin. This works well as a stopgap, but the inescapable fact is that all antibiotics, as presently produced, will eventually succumb to bacterial resistance. The reason is the great ingenuity and promiscuous reproduction of bacteria. Unlike higher creatures such as ourselves, who can only reproduce with their own kind, species of bacteria can exchange genetic information between widely different organisms. Once resistance to a particular antibiotic starts in one organism, it can spread to others by this process of horizontal gene transfer.
A typical story is the developing resistance to carbapenems. These are an important class of antibiotics, related to penicillin and used for severe, high-risk infections. In 2000, a carbapenem-resistant strain of Klebsiella pneumoniae was found in a North Carolina hospital. Until that point, carbapenems were thought to be safe from bacterial resistance. Between 2003 and 2007, the resistance gene spread throughout New York City. It had also reached Israel, the UK, Sweden, Italy and Colombia by 2005. In 2008, another resistance gene was found, this time in Sweden, and its origin traced back to Delhi, India.
We can see this pattern thanks to our enhanced capacity to track infections and to sequence new mutations in bacterial genomes – but our ability to treat these new resistant organisms lags behind. There are many reasons why antibiotic resistance has got out of control, some of which relate to us rather than the bugs themselves. For individuals, if you do not finish a course of antibiotics, a reservoir of bacteria remains that can potentially develop resistance.
For doctors, inappropriate prescription is the problem. Antibiotics are generally dispensed without knowing the precise organism causing the illness. This means that the treatment is often useless, and merely encourages some bacteria to develop resistance. One of the most urgently needed interventions is a cheap, robust way of identifying causative organisms. In some countries, antibiotics are used for the entirely illegitimate reason that they improve the meat yield of farm animals. This has been banned in the EU since 2006 but antibiotic resistance is a global problem; too many countries are still dosing animals for weight gain.
We need a more fundamental change. Antibiotics were the bluntest of instruments when they were introduced. They worked but we didn’t know why; penicillin was introduced nine years before we even knew what DNA was. But since genomics (the science of the structure, function, evolution, mapping and editing of genomes) began to reveal the deep biological processes of life we have learned that bacteria and phages – along with the larger organisms, fungi – are mutually engaged in a complex arms race. With antibiotics we exploited the means fungi developed to ward off bacteria: producing chemicals that kill off bacterial infections before they have a chance to seriously damage their host. Phages work differently: the smallest and most primitive of the three organisms, they are viruses whose raison d’être is to get inside a bacterium’s cell wall, hijack its genes to make more phages, then to burst out and exit to infect other bacteria.
Phages were discovered in 1915 by the English bacteriologist Frederick Twort, who stumbled upon an agent that prevented the growth of Staphylococcus aureus whilst itself reproducing only in the presence of the bacteria. The discovery only came to light two years later when phages were independently discovered by the French-Canadian microbiologist Felix d’Herelle. They are now thought to be the most abundant organisms on Earth, more numerous than all other organisms combined. Phages are parasites made up of protein and DNA, with a precise, geometrical structure that resembles human technology: one variety, the T-series, looks like a lunar landing module. One of their most spectacular properties is their ability to reassemble themselves spontaneously when broken up in a blender.
Phages are our potential ally, because they know more about bacteria than we do. For a phage a single species of bacteria is its whole universe and ecosystem. Phages are everywhere in the environment: outside in swamps and sewage outflows and inside other organisms, including ourselves, where they live in large numbers in our guts. Such phages are harmless to us: they are only concerned with the bacteria they are programmed by evolution to live on.
The first use of phages to treat human beings was in 1919, when D’Herelle tried giving them to dysentery patients. The treatment was successful and so began a curious, almost 100-year history, in which phages have demonstrated their efficacy yet have failed to convince Western medical authorities who insist on the protocols of the controlled clinical trial. Phages, being mutable biological organisms, do not easily fit into a regulatory framework that has been built around one-size-fits-all drugs made from pure, single chemicals. Nevertheless, traditional phage therapy has been safely and successfully used on many patients leading up to Patterson’s miraculous cure.
Traditional phage therapy was used for many decades in the former Soviet Union, with the Eliava Institute at Tbilisi in Georgia as the main centre of expertise. When the Soviet Union collapsed in 1991, the Eliava Institute needed to look outwards. In 1998, Colin Marr, a British technology consultant working for the EU in Georgia, was approached by the Eliava Institute. He was sufficiently encouraged to try and solicit interest in phage therapy from Western pharmaceutical companies. Most replied with a brusque “not interested”, but two of the biggest gave their reasons. Zeneca (now Astra-Zeneca) said, “Production issues, quality assurance difficulties and specifications make this unattractive to us.” GlaxoWellcome gave a very extensive list of objections: the difficulty of bringing this therapy under Western regulations, particularly the US Food and Drugs Administration; the lack of clinical trials; bacterial resistance; the danger of phages themselves spreading resistance genes, and the impossibility of obtaining patent protection for therapies already in use.
In 1993 Alexander Sulakvelidze, from the Georgian phage school, emigrated to the US. On arrival he found an instant use for his phage expertise: resistance to the antibiotic vancomycin was appearing in strains of Enterococcus bacteria. In 1993, working with Glenn Morris at the University of Maryland Medical Center, Sulakvelidze founded Intralytix, now one of the leading phage companies. Despite encouraging results, by 2002 it was obvious that the barriers to regulatory approval for phages in humans would take a long time to surmount, so Intralytix moved into the food industry. In 2006 they developed Listshield to target Listeria monocytogenes, a dangerous bacterial contaminant found in many foodstuffs. The product was the first phage preparation to be licensed for use on human foodstuffs. Other products have followed and this is now an established commercial use of phages.
Phages in food can prevent food-borne disease such as Salmonella and Listeria, but the desperate situation with existing antibiotics, and the genomics revolution, are giving phages a new push in the fight against infections. Patterson’s cure was a bespoke process: a one-off resistant bug was conquered after an extensive trawl through a swamp. For phages to be truly effective against disease, we would need to develop an off-the-shelf phage therapy, tested by clinical trial and licensed, that can be used every time a bacterial organism shows resistance.
The genomics revolution is showing us the precise mechanisms by which phages and bacteria interact. Crucial to many of the approaches is the gene editing technique CRISPR. Bacteria and phages have been at war for perhaps 1.5 billion years and, yes, bacteria can develop resistance to phages too. But since 2012 researchers have been uncovering the detailed mechanism that bacteria use to achieve this and adapted it so that they can turn it back against the bacteria.
The bacterial CRISPR system has two parts: over time, the bacteria develop the ability to “recognise” portions of the phages’ DNA. Whenever they then encounter this signature portion, they dispatch an enzyme which cuts the phage DNA, thus disabling it. If that was all, it would be fascinating. But researchers have been able to program CRISPR to recognise any portion of DNA, cut the molecule at that point and then insert different DNA. There are other gene-editing tools but CRISPR, which is still being refined, is the best.
This detailed knowledge of phage-bacteria interactions has guided researchers to identify parts of bacteria that might be attacked by phage preparations in a way that would make resistance impossible. There are some bacterial functions that cannot mutate without killing the bacterium, and this is where the phage developers want to hit them. There are numerous ways of doing it. Udi Qimron at Tel Aviv University is developing a subtle way of sidestepping the problem of getting regulatory approval for phage use in human beings: his team is developing phage preparations that, used in hospital sprays and cleaning solutions, would make dangerous bacteria susceptible to antibiotics once more. Once inside the bacterium, the CRISPR package is programmed to destroy common antibiotic resistance factors, rendering the bacteria susceptible once more to standard antibiotics. It also protects bacteria that are still sensitive to antibiotics, through a different kind of phage that lodges like a sleeper in the DNA. When a spray of both the CRISPR package and a sleeper phage is applied to bacteria on, say, a hospital surface, or on the skin, the result will be that antibiotic-sensitive bacteria are protected and resistant ones are destroyed. Qimron has patented this approach and set up a company to develop it commercially.
Timothy Lu, at the Massachusetts Institute of Technology, has also used CRISPR technology to target resistance. He has shown that in combination with existing antibiotics,his guided DNA vehicles, delivered by phages, are up to 20,000 times more effective in destroying resistant bacteria than the antibiotic alone. However, questions remain about using such agents in the body (as opposed to Qimron’s external use on surfaces) because phages are large molecules and can provoke a response from our own immune systems, which could be harmful to us.
Vincent Fischetti at Rockefeller University, New York, has developed an impressive array of approaches. Fischetti’s CF-301 is derived from a phage and attacks the bacterial cell wall, usually killing the organism within 30 minutes. It has been effective in trials on mice and is now under clinical trial in a hospital, to treat the common antibiotic-resistant MRSA bacteria. In this double-blind, placebo-controlled study, patients will receive either a single intravenous infusion of CF-301 or a control, along with standard drugs. The results will be published this year. Fischetti’s lab has also managed to screen for a phage therapy that would work on the organism that almost killed Patterson: Acinetobacter baumannii. This is now being developed commercially for use on specific cases of infection.
Phages offer a way beyond the impasse of antibiotic resistance, because of the precision of the interactions between them and bacteria. Scientists can engineer these interactions to target bacteria in a way that antibiotics can’t: to disable vital portions of their DNA. Of the various approaches taken by scientists such as Fischetti, Qimron and Lu, no one knows which, if any, will become mainstream therapies. But phages, these most curious organisms that exist at the very threshold of what is living and what is not, will very likely be part of the solution.
Published 4 April 2019
Original in English
First published by New Humanist 1/2019
Contributed by New Humanist © Peter Forbes / New Humanist / Eurozine
PDF/PRINTSubscribe to know what’s worth thinking about.
Clock times and social times conflict across much of Europe. Timekeeping is a deeply political matter, and João Lipinsky Nunes of Better Times takes issue with them.
The Active Amputee blog’s Björn Eser believes that the way we go about prosthetics should change. He’s a lover of the outdoors, even more so since his amputation, but he takes issue with the limits of social and medical support for disabled people.